What is biophysical economics?
IN SHORT:
Biophysical economics is the study of the ways and means by which human societies procure and use energy and other biological and physical resources to produce, distribute, consume and exchange goods and services, while generating various types of waste and environmental impacts. Biophysical economics builds on both social sciences and natural sciences to overcome some of the most fundamental limitations and blind spots of conventional economics. It makes it possible to understand some key requirements and framework conditions for economic growth, as well as related constraints and boundaries.
Learn more about:
- What biophysical economics is not
- Why biophysical economics matters to policy makers and civil society
IN MORE DETAIL:
Conventional economic ‘science’ analyzes the economy on the basis of a limited set of functions and parameters that are internal or ‘endogenous’ to the economic process. In fact, ‘mainstream’ economics typically envisions the economy as the process of producing goods or services using essentially two ‘factors of production’: capital and labor. Ever since the advent of what we now call the ‘Classical Model’ of the economy, economists have been viewing the production of economic output and its evolution as a ‘function’ of capital and labour, i.e. as resulting from the combination of those two factors and how this combination evolves over time. Economic growth, in this perspective, essentially results from the expansion of the supply of labor and capital inputs and from the rise of the productivity of their use. Labor and capital are the only factors that limit or determine the patterns of scarcity, and most other inputs into the economic process are considered as ‘exogenous’, secondary and largely immaterial to the long-term trends of the economy.

Figure 1: The circular flow of economic activity. The circular flow is a model of the economy in which the major exchanges are represented as flows of money, goods and services, and factors of production (capital, labor, land) between economic agents (individuals/households and businesses). The flows of money and goods exchanged in a closed circuit correspond in value, but run in the opposite direction. More elaborate versions of the circular flow model integrate the government sector, imports and exports, as well as the financial sector. The circular flow analysis is the basis of national accounts and hence of macroeconomics.
This conception, which remains widely prevalent among economists, is however inconsistent with the fact that economic activity is never, in the real world, a closed circular system in which production could be a simple function of capital and labor. A number of other important inputs also need to be brought to the economic process in order for goods and services to be produced, as well as distributed and consumed. By ignoring these other inputs or minimizing their contribution, mainstream economics probably fails to grasp some of the fundamental aspects of how the economy really works.
The most important input into the economic process whose role is commonly overlooked or misunderstood by economists is energy. Far from being a secondary input as most of them assume, energy is a fundamental input that is needed for all human activities, without exception. In fact, capital and labor are functionally inert without energy input. It is only through the use of energy that they can be combined to produce and distribute goods and services, and the productivity of their use therefore depends to some extent upon the quantity and quality of this energy input.
The centrality of energy to all forms of human activity is often obscured by the fact that energy comes from various sources and is used in various forms that are described and measured in different units. Energy is contained in our food, in gasoline or in stars, it moves from one form to another via wind, motion and heat. Energy is a word with many meanings yet no universal definition, something that is both intuitively obvious yet abstract and complex. However, all forms of energy are fundamentally dimensions of the same thing: the capacity of a physical system – be it a human or animal body, a car, plane, computer, factory, power grid, economy, etc. – to perform work and/or to transform matter. Obtained through food calorie intake, this capacity enables human beings to perform a number of physical and intellectual activities. Obtained through other sources of energy, it enables various types of machines to perform a wealth of activities that go far beyond the capability of human or animal muscles and brains.
Without energy/capacity to perform work and/or to transform matter, there can be no human activity and hence no economy, and without development of this capacity there can be no expansion of human activity and of the economy. Achieving economic growth thus typically requires procuring and using increasing quantities of energy. In fact, economic growth has historically been closely correlated with the capacity of the countries and regions that experienced it to harness and use ever-growing amounts of energy.
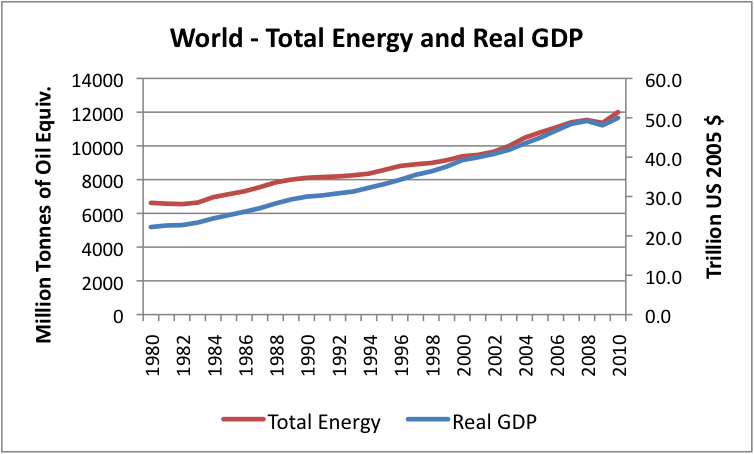
Figure 2 : Growth in world energy consumption (based on BP data) and growth in world real GDP (based on USDA Economic Research Institute data), 1980-2010. Source: Gail Tverberg, Ourfiniteworld.com
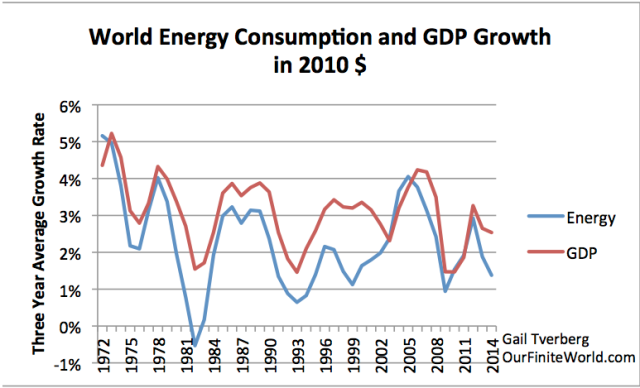
Figure 3: Three-year average growth rate in world energy consumption and in GDP, 1972-2014. World energy consumption data based on BP Review of World Energy 2015; real GDP from USDA in 2010$. Source: Gail Tverberg, Ourfiniteworld.com

Figure 4: Correlation of energy consumption and GDP per person. The graph shows per capita energy consumption (kg oil equivalent) vs. per capita GDP, PPP (current international $). The size of the bubbles denotes total population per country. All values refer to the year 2011. Source: European Environment Agency, 2014.
In order for goods and services to be produced, distributed and consumed, energy needs to be combined with other inputs – labor and capital inputs, of course, but also various types of matter, i.e. biological and physical substances obtained, directly or indirectly, from natural resources such as land, water, soil, plants, animals, and minerals. The relation between the use of these biophysical resources (including energy resources) and the economy is however complex and difficult to apprehend. Most economists tend to see energy and resource use as mere consequences of the economic process, but a growing body of research suggests that the relation is more complex and consequential than commonly assumed. Nevertheless, as a result of mainstream economics’ relative disregard for the role of energy and biophysical resources, of the resulting lack of appropriate and objective data collection and maintenance, as well as of insufficient support for related research, the nature and extent of the contribution of energy and matter to the economic process, and hence to economic growth, remain insufficiently understood and largely overlooked.
Biophysical economics aims to conceptualize and quantify this contribution. To this end it puts energy where it belongs, i.e. at the centre of the economic process, and analyzes how the flows of energy and matter shape and are shaped by the economy’s structures and evolutions. It is based on a number of key principles:
- The economy is a complex, ‘dissipative’ system
- Economic processes are ‘metabolic’ in nature
- Energy sources and uses impact economic structures and performances
- Energy sources and resources are not perfectly or easily substitutable
- Energy sources historically tend to supplement rather than replace each other
- Economic growth cannot be fully decoupled from increasing energy use
- ‘Energy quality’ varies between resources and over time and space
- The ‘net energy gain’ of energy resources and systems varies over time and space
- Energy gets ’embodied’ in all goods and services produced and exchanged
- Energy, money and finance are interconnected
- The flows of energy and matter are interdependent
- Non-renewable biophysical resources are exhaustible and subject to depletion
- The use of biophysical resources induces environmental degradation
The economy is a complex, ‘dissipative’ system
The economy is not a simple and closed circular system, but a complex system whereby energy and matter get procured from various sources in the environment and then converted and used – in combination with other inputs, including labor and capital – to produce, distribute and consume the goods and services that provide sustenance, security, comfort, mobility and entertainment to human beings, creating various types of waste at all stages. In other words, the economy is a ‘dissipative’ system, i.e. a thermodynamically open system that ‘exchanges’ energy and matter with its surroundings. As a consequence, economic activity is necessarily constrained, at global level, by the laws of thermodynamics, i.e. the inescapable physical laws that govern how heat (or thermal energy) is converted to and from different types of energy, and the effect that this can have on various forms of matter. These laws in fact imply that human beings can never create energy but only harness it from sources present in nature, and that their use of energy sources present in nature always generates an irreversible dissipation/degradation of energy, from ‘usable’ to ‘unusable’, and a parallel increase of entropy, i.e. systemic randomness or disorder, in particular in the form of environmental degradation.

Figure 5: Energy and the economy – adapted from Charles A.S. Hall
Economic processes are ‘metabolic’ in nature
The economy can be viewed as a set of metabolic processes, i.e. processes by which human societies – and their various components – ‘exchange’ energy and matter with their biophysical environment and between themselves, and use them in various ways and for various purposes. These metabolic processes vary considerably in time and space according to the internal structures of societies and economies, to their ‘metabolic patterns’ (i.e. the patterns of energy and resource use by their various components and constituents) as well as their trade patterns.

Figure 6: Society’s material and energy flows. Source: Haberl et al 2004
Energy sources and uses impact economic structures and performances
Primary energy sources take many forms, including nuclear energy, fossil energy (oil, coal and natural gas) and renewable sources such as wind, solar, geothermal and hydropower. How energy is procured and used in a given society or geographical region depends on the availability of usable energy resources on its territory or the possibility of importing them, the extent and type of energy needs to be met, as well as the characteristics of its energy system(s), which are influenced by historical, economic, social, demographic, environmental and geopolitical factors. Energy supply and use are thus influenced by a society’s patterns of production and consumption, but they also contribute to shape them and to support or constrain their evolutions.

Figure 7: World primary energy consumption (in million tonnes oil equivalent) and primary energy consumption by fuel and by region (in percentage), 2015. Oil is the world’s dominant fuel and gained global market share for the first time since 1999, while coal’s market share fell to the lowest level since 2005. Renewables in power generation accounted for a record 2.8% of global primary energy consumption. Oil remains the dominant fuel in Africa and the Americas, while natural gas dominates in Europe & Eurasia and the Middle East. Coal is the dominant fuel in the Asia-Pacific region, accounting for 51% of regional energy consumption – the highest share of any fuel for any region. Europe & Eurasia is the only region with no fuel reaching one-third of the total energy mix. The Middle East has the least diverse energy mix, with oil and gas combined accounting for 98% of energy consumption. Source: BP Statistical Review of World Energy 2016.
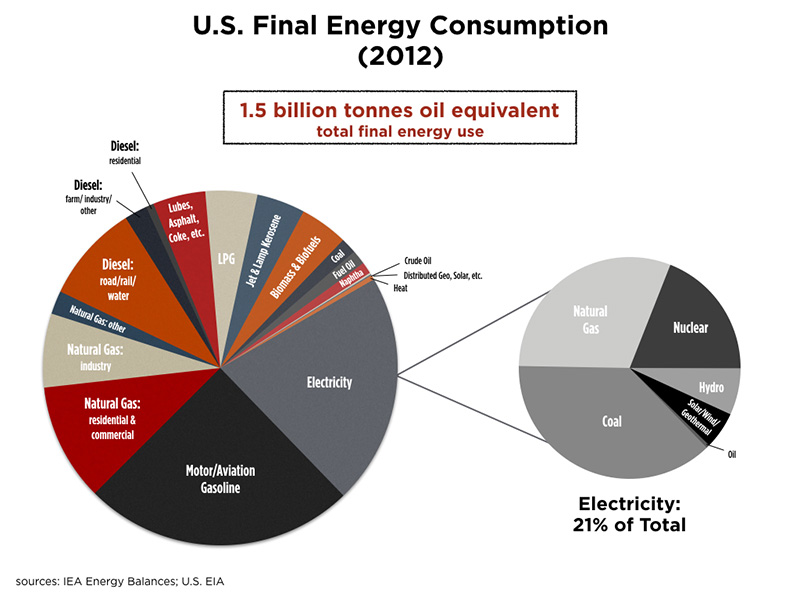
Figure 8: U.S. Final energy Consumption 2012. Electricity accounts for less than a quarter of all final energy used in the US. Transportation, buildings and the food systems are major energy consumers. Source: Post Carbon Institute. Data from IEA and U.S. EIA

Figure 9: U.S. energy flows in 2014. Distributed electricity represents only retail electricity sales and does not include self-generation. EIA reports consumption of renewable resources (i.e. hydro, wind, geothermal and solar) for electricity in BTU-equivalent values by assuming a typical fossil fuel plant “heat rate”. The efficiency of electricity production is calculated as the total retail electricity delivered divided by the primary energy input into electricity generation. End use efficiency is estimated at 65% for the residential and commercial sectors, 80% for the industrial sector, and 21% for the transportation sector. Totals may not equal sum of components due to independent rounding. Source: Lawrence Livermore National Laboratory (LLNL) 2015. Data is based on DOE/EIA-0035(2015-03), March, 2014.
Energy sources and resources are not perfectly or easily substitutable
The composition and evolution of the primary energy supply – or ‘energy mix’ – impacts the economy in various ways. While mainstream economics views energy as a mere ‘commodity’ – i.e. an easily substitutable marketable item that can be bought, sold, and traded across a market with little or no qualitative differentiation – biophysical economics recognizes that energy comes from various sources and in various forms that have very different physical properties and capacity to generate economic and social value, especially when problems of scale and resource dependency are factored in. Key attributes include availability and affordability, energy density (amount of energy stored per unit volume or mass), power density (rate of energy flux obtained per unit of spatial area used), versatility of use, including fungibility (equivalence and interchangeability of all units), storability (capability to be stored for significant time without loss of usability), transportability (capability to be moved around by multiple means with limited loss), convertibility (capacity to be efficiently converted into other forms of usable energy), and scalability (capacity to be scaled up or down efficiently, cost-effectively and without adverse impact). All of these attributes make fossil fuels, and most particularly petroleum, very convenient, economic, powerful and versatile sources of energy and explain why they have become the master energy sources of the modern world. They also explain why all units of energy are not necessarily equal and interchangeable in an economy at any point in time, and why attempts at substituting dominant energy sources with alternative ones – or even a specific type of energy resource (e.g. conventional oil) by another with different properties (e.g. unconventional oil) may not lead to the intended outcome when net economic effects (including system/infrastructure costs) are considered. In other words, the theoretical potential of an energy source or resource base is not always equal to its economic potential, let alone to its practically realizable potential. Energy transitions are therefore complex and lengthy processes that affect the structures and functioning of the economy in ways that cannot necessarily be understood in purely monetary terms and captured in money-based cost-benefit analyses.
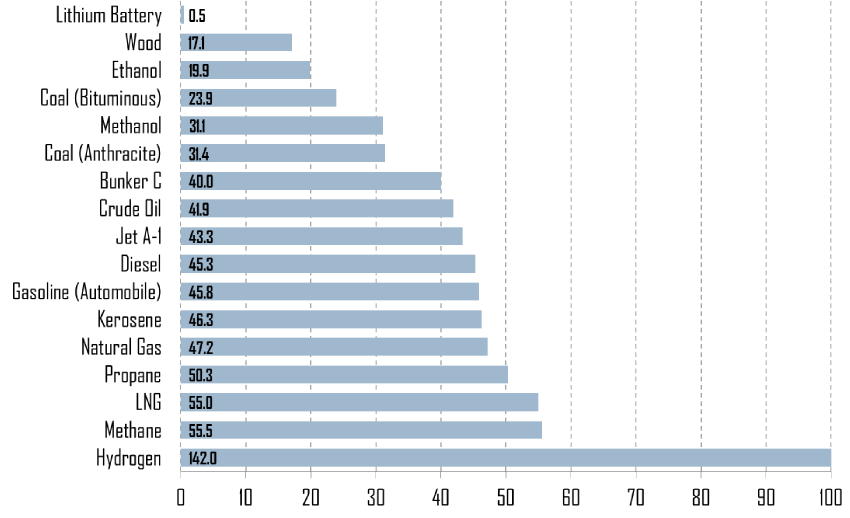
Figure 10: Energy content of some combustibles (in MJ/Kg). Different fuels have different energy densities and the above graph relates to energy released through combustion, in MegaJoules per Kilogram (MJ/Kg). The higher quantity of energy released, the higher the quality of the fuel, which is inversely proportional to its chemical complexity. High quality fuels are gases while low quality fuels are solids, with liquids in between. The fuel with the highest energy content is hydrogen, which is also the simplest chemical component in existence. Gasoline, which is derived from refining crude oil, contains much more energy than coal (twice as much as the lower grade bituminous) or wood (three times). Liquid natural gas (LNG) is almost entirely composed of methane, while natural gas has about 85% of its mass accounted for by methane. Jet A-1 is the standard fuel used by commercial jet planes and is mostly composed of kerosene and a number of additives to make the fuel suitable for use in high altitudes and low temperatures. Bunker C fuel is the main fuel used for maritime shipping and is a lower quality fuel in liquid form, but suitable for large ship engines). Although methane and hydrogen both have higher energy content than gasoline, their gaseous form creates storage difficulties. Furthermore, hydrogen must be synthesized, which requires significant energy investment. One of the most efficient electrical power storage devices, the lithium battery, can only hold about 0.5 MJ per kilogram. Source: Hofstra University.
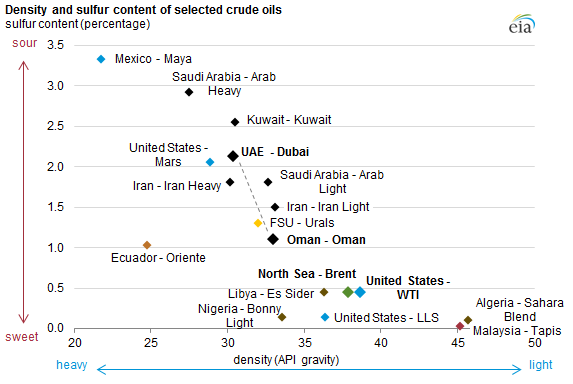
Figure 11: Density and sulfur content of selected crude oils. Many types of crude oil are produced around the world, which have various quality characteristics. Two of the most important characteristics are density and sulfur content. Density ranges from light to heavy based on the American Petroleum Institute (API) gravity formula, while sulfur content is characterized as sweet and sour. Crude oils that are light and sweet are usually considered as better quality and hence preferable than heavy, sour ones. The light sweet grades can indeed be processed with far less sophisticated and energy-intensive processes/refineries. These different characteristics imply an imperfect substitutability between different types of crude oil, meaning that an aggregate analysis of the crude oil market often tends to be misleading.
Energy sources historically tend to supplement rather than replace each other
Historically, new energy sources have always, at global level, supplemented rather than replaced or substituted preexisting ones. Even if coal supplanted water power and petroleum supplanted coal as the world’s dominant energy source in relative terms, they never replaced them in absolute terms. Instead, coal was added to the existing and growing fund of water power, and petroleum was added to the total when it became available. Since then, natural gas and nuclear power have been added to the global energy mix as well, and “modern renewables” (wind and solar power) are now being added to already existing energy sources without, so far, replacing any of them at global level – even if a limited partial replacement may occur at national/regional level in some cases. All these energy sources add up to each other to meet a total energy consumption that has been rising since the Industrial Revolution and that keeps rising. The expected partial or total replacement this century of fossil fuels by “modern renewables”, in absolute terms, would therefore constitute a systemic change without any precedent in human history, which would profoundly impact the structure and functioning of the global economy in multiple ways.

Figure 12: Development of the world’s total energy consumption split by source from 1800 to 2013. Source: Nate Hagens and Rune Likvern, Fractional Flow
Economic growth cannot be fully decoupled from increasing energy use
The economic value and contribution of the energy supply depends on its composition, but also on its metabolic pathway, i.e. how efficiently primary energy gets converted by the various components of an energy system (i.e. various types of power plants, solar panels, wind farms, etc.) into usable ‘secondary energy’ sources or ‘energy carriers’ (i.e. electricity, fuel for transportation, natural gas), which in turn get transported and distributed to the point of use where they provide ‘energy services’ (e.g. heating, cooling, lighting, mechanical power, and electricity) to consumers, businesses and governments. Those energy services are then used to produce a wealth of private and public goods and services. It is widely acknowledged that the efficiency of energy use at all these stages can be raised, in particular through technological progress and system design, making it possible to produce more goods and services per unit of energy used, i.e. to increase the ‘energy productivity’ and decrease the ‘energy intensity’ of the economy. However, the potential for energy efficiency gains is bounded by physical laws as no energy-using economic process can take place without dissipation and loss of energy. In addition, historical evidence shows that investments in energy efficiency are subject to the law of diminishing returns – meaning that the financial and energy investments needed to achieve a given amount of efficiency improvement tend to rise over time – and that energy consumption is subject to a so-called ‘rebound effect’ (also called ‘Jevons Paradox’) – meaning that efficiency gains in the use of a given resource tend to increase rather than decrease its rate of consumption. As a consequence, there are limits to how much economic growth can be ‘decoupled‘ from increasing energy use and from resulting environmental impacts.

Figure 13: Energy efficiency and the proliferation of electronic gadgets – an illustration of the ‘Jevons Paradox’. Source: The New York Times, September 2009
‘Energy quality’ varies between resources and over time and space
Only part of the total energy input can actually be converted into ‘useful work’ that can effectively contribute to the economic process – in the form of muscle work, mechanical and electrical power, and heat delivered to the point of use. Some of the raw energy input is indeed either too low quality to do actual work or gets degraded or wasted in the process of conversion, transformation and transport. Physicists and engineers call ‘exergy’ the energy that is effectively available to produce ‘useful work’ in a system. Only ‘useful exergy’ – i.e. exergy made available at the use stage and after transformation and conversion – effectively serves to provide ‘energy services’. Different energy resources have different capabilities to generate work (and hence different amounts of exergy), and therefore different relative economic usefulness per unit. The ratio of exergy to energy in a substance or an energy resource can thus be considered as a measure of ‘energy quality’. Research shows that the increased availability of cheaper and higher quality forms of exergy inputs, and the rising efficiency of their conversion to useful work, have historically played a key role in driving productivity and economic growth in industrialized and emerging economies.
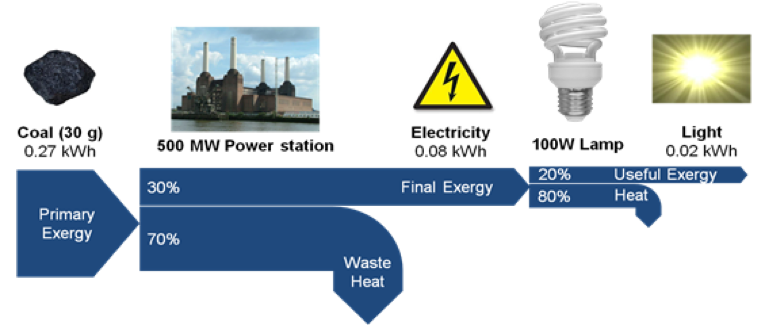
Figure 14: Conversion of primary exergy to useful exergy: primary-to-final-to-useful exergy conversion stages for illustrative lamp (courtesy of T. Domingos, Instituto Superior Tecnico, Lisbon)
The ‘net energy gain’ of energy resources and systems varies over time and space
The procurement and delivery of energy to the economy is a process that itself requires using significant amounts of energy, meaning that only part of the total energy supply can effectively be used for doing other things than finding, extracting, processing, converting, transporting and distributing energy. This amount is sometimes called ‘net energy’ or ‘surplus energy’. Different energy resources have different capabilities to generate net energy, which impacts their relative economic usefulness for society. A metric often used to measure the net energy ratio of various types of energy resources is the ‘energy return on (energy) invested’ (abbreviated EROI or EROEI) i.e. the ratio between the total amount of energy delivered by an energy resource during its working lifetime and the amount of energy invested in obtaining and delivering it. Net energy is difficult to measure, and EROI calculations can vary significantly depending on the accounting methods and boundaries used and on whether corrections for energy quality are made. In addition, the net energy returns of certain energy resources can evolve in a non-linear way depending on a variety of factors including technological progress, production pathways and change in resource base. The economic value of net energy delivered by a given resource at the point of use may also vary in time and space depending on system/infrastructure costs and on the various potential uses of energy. Most analyses nevertheless show that the net energy gain of fossil fuels is relatively high but decreasing over time as resources get depleted, remaining resources decrease in quality and get more difficult to extract, whereas alternative energy resources tend to have lower net energy ratios that can in some cases rise over time with technological advancement. Research suggests that the increased availability of high net energy resources – i.e. fossil fuels, and most particularly oil – has historically represented a key driver of economic growth as well a key enabler of growing economic and societal complexity as the resulting surplus energy available to society made it possible to develop a wealth of activities that would not have been otherwise possible.
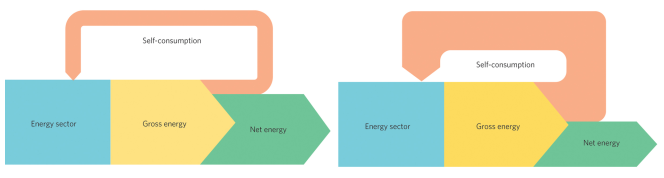
Figure 15: Net Energy Analysis (NEA) studies the net output of energy-producing technologies, accounting for the energy consumed, directly and indirectly, by the energy sectors, in contrast to the gross energy production measured by the International Energy Agency (IEA) and the US Energy Information Administration (EIA) in their analyses. Source: A better currency for investing in a sustainable future, by Michael Carbajales-Dale, Charles J. Barnhart, Adam R. Brandt & Sally M. Benson, in Nature Climate Change 4, 525-527 (2014)
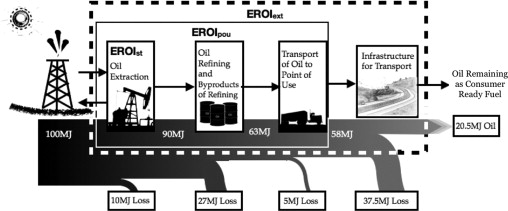
Figure 16: Boundaries of various types of Energy Return on Investment (EROI) analyses and energy loss associated with the processing of oil as it is transformed from “oil at the well-head” to consumer ready fuels. Based on calculations by Hall et al, 2009.
Energy gets ’embodied’ in all goods and services produced and exchanged
Energy gets ’embodied’ into all goods and services produced, in direct and indirect ways. ‘Embodied energy‘ can be understood as the sum total of the energy necessary to make goods and services, from extraction to production. It may also be accounted for, however, over the entire product life-cycle, including raw material extraction, transport, manufacture, assembly, installation, distribution, disassembly, deconstruction and/or decomposition, as well as secondary resources and the energy costs of labor and government services. The concept of embodied energy can be useful to assess the effectiveness of energy-producing or energy-saving devices as it allows to compare the amount of energy produced or saved by the product in question to the amount of energy consumed in producing, operating and disposing or decommissioning it. Embodied energy is also useful to determine and analyze the flows of energy between various societies, countries, or social groups as these flows are not limited to energy products but also include the energy embodied in the goods and services they exchange with others.
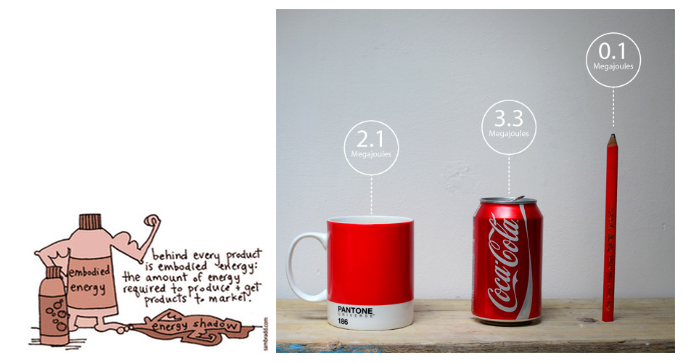
Figure 17: Embodied energy – Estimates of how much energy it took to manufacture three simple consumption products. Source: productdesignhub.com

Figure 18: World energy trade of total direct energy (black) as well as embodied energy in goods traded (grey). The literature and statistical basis of embodied energy flows is thin, as embodied energy trade is quite under-researched and not reported systematically. The only data source available for estimating embodied energy is the Global Trade Analysis Project (GTAP) database that contains data suitable for estimating the fossil fuel energy embodied in international trade flows by input-output analysis. Important limitations need to be considered when trying to estimate the energy embodied in internationally traded commodities and products, and the flows summarized in the figure above need to be considered as orders of magnitude estimates that await further analytical and empirical refinements. Nonetheless, this data helps to get a sense of proportion. GTAP estimates that (fossil) energy embodied in international trade amounts to some 20% of global primary energy use, and shows that many industrialized countries are net importers of embodied energy, while many developing countries are net exporters. Embodied energy trade flows illustrate the multitude of interdependencies at play in the global energy system, that go far beyond traditional concerns of oil or gas import dependency, and provide a broader perspective for studying relationships between energy-producing and energy-consuming regions. Source: Global Energy Assessment, 2012. Data sources: embodied energy trade for 2004 – GTAP database, 2010; direct energy trade – BP, 2007, WCI, 2009.
Energy, money and finance are interconnected
As energy gets ’embodied’ in direct and indirect ways into all goods and services produced and consumed, it therefore plays a crucial role in the process of value creation across the whole economy. Money, which is the standard measure of value in modern economies, may be viewed as a ‘claim on energy’, i.e. a token representing a claim on a certain amount of biological and physical work, usually already done, that was accomplished through the use of energy. Similarly, credit can be understood as a claim on future biological and physical work, to be accomplished through the future use of energy and therefore dependent upon the availability and quality of future energy resources. The monetary value of a good or service in market transactions may of course differ from its energy cost, but research shows that over the long term there is a strong statistical relation between economic value and embodied energy content if energy calculations include, in addition to direct energy use, an estimate of the quantity of energy indirectly used in production (e.g. energy costs of labor and government services) as well as of unaccounted ‘externalities’ (e.g. environmental degradation or resource depletion). Therefore, there is a connection – even if a loose one over the short term – between energy flows and money flows, which tend to move in opposite directions, and between a society’s energy metabolism and its financial system. Over the long term, factors affecting the metabolic pattern of a given society may have a significant impact on the stability and sustainability of its financial system, and factors affecting the financial system may have an impact on energy provision and use, especially as energy and commodity markets get increasingly ‘financialized’ (i.e. subjected to the risk appetite and returns expectations of financial investors, rather than just to supply and demand fundamentals).
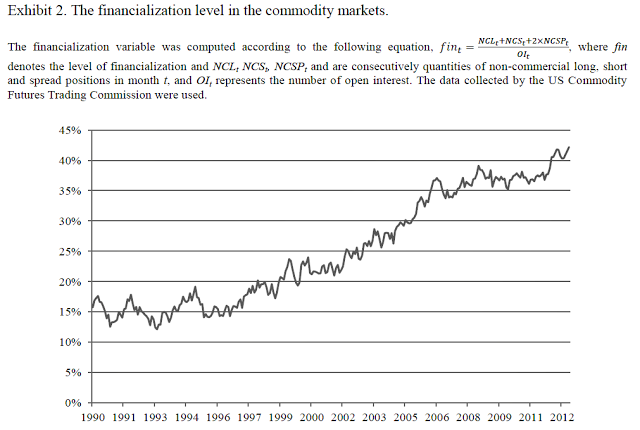
Figure 19: Financialization level in the commodity markets. Financialization is defined as the share of all ‘non-commercial’ traders’ positions in the market (NCL: non-commercial longs, NCS: non-commercial shorts, NCSP: non-commercial spreads). The share of non-commercial (i.e. financial) traders in the commodity markets has systematically increased over the last 20 years at a more or less even pace, starting from 15% at the beginning of the 1990s to over 42% at the end of 2012. Source: Is Financialization Killing Commodity Investments? By Adam Zaremba, Poznan University of Economics. In Journal of Alternative Investments, Vol. 18, No. 1, 2015, pp. 66-91.
The flows of energy and matter are interdependent
As a master resource for human activity, energy affects the production and use of all other biological and physical natural resources that are also required for goods and services to be produced, distributed and consumed in modern economies (e.g. plants or plant-based materials, water, minerals, etc.). The provision of those material resources may in turn impact the quantity and quality of the energy supply. Therefore, a certain degree of interdependence exists between the availability and use of energy resources and the availability and use of other natural resources, to the point of constituting a ‘nexus’ in some cases (e.g. the ‘water-energy’ nexus). A close relationship exists, for example, between the energy and metals sectors, as a significant and rising share of metals extracted are used by the energy sector (in particular renewable technologies that require large amounts of matter, including both common and rare metals), and a significant and rising share of energy is used for metal extraction and processing as depletion progresses and mineral ore grade goes down.
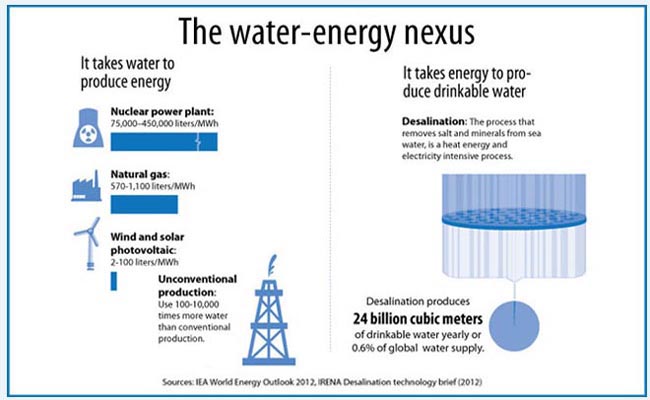
Figure 20: The Water-Energy Nexus. It takes water to produce energy. Renewable energy sources like solar and wind are the most water efficient ways to produce electricity. Energy is also increasingly used to produce freshwater. The International Renewable Energy Agency (IRENA) expects demand for desalination to grow at an annual rate of 9% over the next five years. Source: GE Look Ahead, 2013.

Figure 21: Materials required in the manufacturing and operation of a wind turbine. Source: Post Carbon Institute.
Non-renewable biophysical resources are exhaustible and subject to depletion
A number of biological and physical natural resources – including energy resources – are non-renewable (or only partly or slowly renewable), and therefore stock-based and exhaustible. As their use increases their reserves are subject to a phenomenon of ‘depletion’, which tends to render them scarcer, degrade the quality of the resources obtained, and make their procurement (extraction or harvesting) more expensive and resource-intensive over time – which may in some cases end up making their use uneconomic (i.e. unprofitable). Mainstream economists view resource depletion as an ‘externality’, i.e. an element that is external to the economic system and for which producers and consumers bear no direct costs. They typically deny that resource depletion may impose constraints on the ability of the economy to expand, as they argue that the price system and appropriate market mechanisms and/or government intervention can ensure that depleting resources get ‘substituted’ in due time by other near-equivalent natural or man-made resource(s) in order to satisfy demand. Biophysical economics on the contrary recognizes that the principle of substitutability only partially applies to exhaustible natural resources (including exhaustible energy resources), and that replacement resources may not be able to provide the same services and value to society or only with increasing price and/or energy use. Attempts at substituting critical resources may thus impact the economic system’s ‘metabolism’ and growth potential in various and complex ways.

Figure 22: Stock Check – Estimated remaining world supplies of non-renewable resources. Source: BBC, June 2012
The use of biophysical resources induces environmental degradation
The extraction, transformation, transport and use of biological and physical natural resources – including energy resources – as well as the production, distribution, consumption and disposal of goods and services that they make possible, generates various types of ‘waste’ or ‘pollution’, which are returned to the biological and physical (i.e. biophysical) environment. This use of the environment as a ‘sink’ for waste energy and matter often tends to negatively impact the biophysical ecosystems from which these resources are obtained, and can in some cases weigh on society’s capacity to extract or use them. In conventional economics, environmental degradation – including climate change – is considered as an ‘externality’ for which producers and consumers bear no direct costs. Economists typically assume that the price system and appropriate market mechanisms and/or government intervention can ensure that environmental degradation gets minimized or corrected. Biophysical economics on the contrary recognizes that the degradation of the biophysical environment resulting from the economic process – and hence from economic growth – conveys costs that do not appear in business or national accounts but that are significant and growing over time and may end up weighing on the capacity of the economic system to develop and expand, or even to sustain itself.
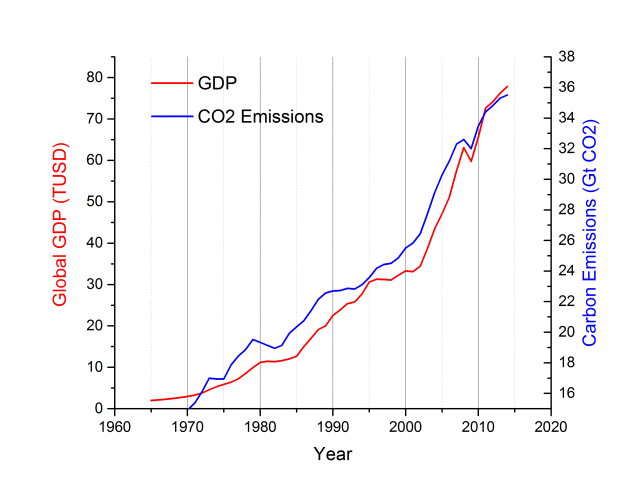
Figure 23: Global CO2 emissions and GDP from 1965 to 2014. No decoupling between economic growth and carbon emissions is observable at global level, even if some decoupling seems to be occurring in some advanced economies. Source: Global Initiative for a Sustainable Economy (GISEco). Data on CO2 emissions from the Carbon Dioxide Information Analysis Center and from BP. Data on GDP from the World Bank.
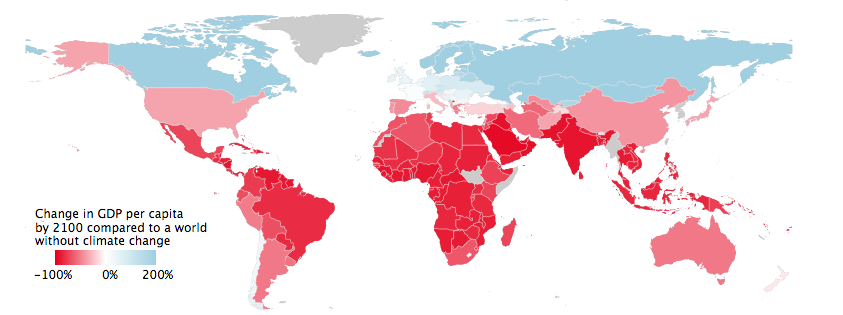
Figure 24: Estimated change in GDP per capita by 2100 compared to a world without climate change. Source: Global non-linear effect of temperature on economic production, by Marshall Burke, Solomon M. Hsiang, and Edward Miguel, published in Nature, November 2015. Differences in the projected impact of warming are mainly a function of countries’ baseline temperatures, since warming may contribute to raise productivity in cool countries. At global level the authors estimate that climate change may reduce projected global output by 23% in 2100 relative to a world without climate change, although statistical uncertainty allows for positive impacts with probability 0.29
Building on those principles, biophysical economics provides an alternative view of how the economy works, grounded in biological and physical reality and different from the view commonly admitted and propagated by conventional economics. It makes it possible to understand how energy contributes to the economic process and to economic growth – a contribution that goes well beyond the ‘cost share’ of primary energy (i.e. the level of primary energy expenditures as a share of GDP) that is commonly considered in conventional economics. In fact, a growing body of research suggests that the relation between energy use and output growth, rather than a simple correlation, might be one of co-integration (meaning that the two tend to converge over a relatively short period of time), and that the direction of causality might not be as straightforward as commonly assumed by most economists. Energy might in fact underpin a large part of the ‘Solow residual‘, i.e. the ‘residual’ economic growth that economists cannot explain through capital accumulation or increased labor, which is historically the larger part of economic growth and that they tend to attribute to difficult-to-quantify ‘technological progress’ or ‘total factor productivity‘.
Biophysical economics therefore makes it possible to better understand the framework conditions for economic growth, as well as corresponding constraints and boundaries. It also makes it possible to better understand the key requirements for societal sustainability, and sheds a different light on past economic developments as well as on current and future economic prospects and challenges.
Learn more about: